Modeling Groundwater Denitrification by Ferrous Iron with PHREEQC
Tedros Tesfay has a B.S. in Chemistry from the University of Asmara, Eritrea, and a M.S. in Radiogenic Isotope Geochemistry from University of New Mexico, Albuquerque, New Mexico. He is currently pursuing his Ph.D. in Geology at the University of North Dakota with a research specialization of hydrogeology.
Fellow: Tedros Tesfay, Department of Geology and Geological Engineering, UND.
Advisor: Scott Korom, Professor of Geology, UND.
Matching Support: In-kind contribution from Dr. Korom’s salary, waiver of indirect costs, a graduate tuition waiver
Degree Progress: Ph. D. expected in 2005.
Modeling Groundwater Denitrification by Ferrous Iron with PHREEQC
Problem Statement: Elevated concentrations of nitrate cause some health problems such as methemoglobinemia in infants (Afzal, 2006), while the relationship between ingested excess nitrates and deadly diseases, such as stomach cancer and negative reproductive outcomes in adults are debatable (Manassaram et al, 2006). Once groundwater is contaminated, the cost of protecting consumers from excess nitrate health-risks is high. Denitrification is the only effective process that converts significant amounts of nitrate irreversibly into harmless nitrogen gas in groundwater environments (Korom, 1992 and references therein). It is a natural process that requires an anaerobic environment, denitrifying bacteria, and sufficient and reactive electron donating species (Firestone, 1982). Numerous studies show that the availability of electron donors limits the denitrification potential of aquifers (Trudell et al., 1986; Korom, 1992; Starr and Gillham, 1993; Robertson et al., 1996). Hence, knowledge of the natural denitrification capacity of aquifers, through the analysis of electron donors, is required to manage the ongoing nitrate load into groundwater systems.
Studies made by members of the University of North Dakota (UND) denitrification research team show that organic carbon and sulfides are active electron donors in North Dakota and Minnesota aquifers (Korom et al. 2005). However, the role of Fe(II) was overlooked (Schlag 1999; Skubinna 2004) and my research complements the previous works by investigating the role of Fe(II) in the regional aquifer denitrification processes. Geochemical modeling, PHREEQC, is employed to gain insight into the in situ denitrification processes that take place via all major electron donors.
Research Progress
All things worked out as planned and as I indicated in my last progress report I have completed successfully the doctoral exam on May 08, 2006. Moreover, results of the research were presented in the 40th annual meeting of the Geological Society of America in Akron, Ohio, on April 21, 2006. I am indebted to the North Dakota Water Resources Research Institute, funded by the United States Geological Survey and the North Dakota State Water Commission (NDSWC), and the North Dakota Department of Health for their financial assistance throughout the project. Currently, I am working on the final edition of the dissertation and will be ready in two-three weeks. There is also a plan to publish results of this research in one or more of the prestigious geological and hydrogeological journals.
Summary of the Research Results
A better way to estimate groundwater denitrification reaction is to compute the mass balance of the redox sensitive species. The University of North Dakota (UND) denitrification team installed mesocosms (ISMs) to understand the fate of a contaminant nitrate in the actual field conditions. Many studies, including the works of the UND denitrification team, have shown evidently, using the mass balance method of estimation, the significant role of sulfides (dominantly pyrite) and organic carbon in the denitrification processes of aquifers. However, the role of Fe(II) has largely been overlooked in the regional studies mainly because of two reasons: 1) the geochemical evidence for ferrous iron is more difficult to decipher due to the precipitation of Fe(III)-oxyhydroxides from the aqueous solution. 2) in the event when denitrification by both Fe(II) and organic carbon gave rise to precipitating reaction products, the role of Fe(II) is deceivably masked by that of the organic carbon. Therefore, two important measures were taken to tackle the problems.
First the abundance of Fe(II) and the minerals that host it were determined using multiple complementary analytical techniques: wet chemical extractions, x-ray diffraction and Mössbauer spectroscopy. The results of these analyses confirmed that the sites, where pyrite and organic carbon did not seem to be dominant, are found to be relatively rich in potential ferrous iron minerals. Then the use of a geochemical modeling resolved the intricacy between the two precipitating denitrification reaction products by figuring out the maximum amount of inorganic carbon that could be produced in the process. First, PHREEQC simulated the amount of inorganic carbon precipitated out from solution indirectly through the co-precipitating Ca 2+ and Mg 2+ that were released into solution by cation exchange reactions. In some of the sites, Ca 2+ and Mg 2+ mmoles/L were also decreased in solution. Therefore, computing the mass balance of Ca 2+ and Mg 2+ provided the maximum fraction of these cations lost from both the solid phase and solution. If all these cations were assumed to be co-precipitated together with the inorganic carbon, which is not likely, it provides the upper limit for the inorganic carbon that can possibly produced in the N-ISMs. By process of elimination the net nitrate lost due to denitrification but not accounted for by reactions with pyrite and organic carbon was attributed to Fe(II) and verified through the forward geochemical modeling.
Figure 1a. Robinson (North Dakota) Nitrate mesocosm: Modeled (dashed lines) vs. Measured (solid lines) Cations-N-ISM.
During the verification work emphasis was given to the modeled and measured cations, anions and pH values. Cations of the Robinson N-ISM matches well (Fig. 1a). As expected Na+, the cation associated with the tracer Br-, shows some deviations. Measured and modeled anions, except some minor deviation in Robinson (Fig. 1b), are in agreement. Recalling the challenge of imitating the natural geochemical environment on one side and practicality of the modeling work on the other side, the above observations are satisfactory.
Figure 1b. Robinson (North Dakota) Nitrate mesocosm: Modeled (dashed lines) vs. Measured (solid lines) Anions-N-ISM (colored results for inorganic carbon and pH represent three different scenarios during the forward geochemical modeling: 1. when net nitrate forced to react with Pyrite, CH2O and Fe(II) (black). 2. When net nitrate forced to react with Pyrite and Fe(II) only (red) 3. When net nitrate forced to react with Pyrite and CH2O only (blue))
Table 1. Relative Roles of the Common Reductants in Aquifer Denitrification Reactions for Akeley (MN), Robinson (ND) and Karlsruhe-S (ND)
Figure 2. Average Contribution of Each Electron Donor in the Natural Denitrification Reactions of North Dakota and Minnesota Aquifers, as Computed via Advanced Geochemical Modeling, PHREEQC; Employing the Concept of Forward Geochemical Modeling (Akeley (MN), Robinson (ND) and Karlsruhe-S (ND)).
Conclusion
All aqueous analytical data, mineralogy and chemistry of sediments and geochemical modeling works are evidently showing the proportional role of the common electron donors (Fig. 2) and Fe(II)-supported denitrification has a significant role as a natural remediation process (Table 1). Validation of the modeling work by comparing output files with the target solutions of different time steps, chosen previously to verify the work, demonstrate that dilution, CEC and reversible reactions were responsible for the geochemical evolution observed in the C-ISM. Whereas for the nitrate chamber, in addition to dilution, CEC, and reversible reactions, denitrification reaction that involves CH2O, FeS2 and Fe(II) were the major processes that evolved the geochemical environment of the N-ISMs. Moreover, close observation of the hydrochemical data of the ISMs also demonstrate that denitrification rate was higher for those sites with high concentration of electron donors and vice versa.
References
Afzal, B. (2006). Drinking water and Women's heath. Journal of midwifery and women health, v. 51, issue 1, 12-18.
Firestone, M. K. (1982). Biological denitrification, in Nitrogen in Agricultural Soils, edited by F. J. Stevenson, American Society of Agronomy, Madison, Wisconsin, 289-326.
Gillham, R.W., and J.A. Cherry, 1978. Field evidence of denitrification in shallow ground water flow systems. Water Pollution Research in Canada 13:53-71.
Kammer, A. E., 2001. Laboratory denitrification using sediments from the Elk valley aquifer, M.S. thesis, 76 pp., University of North Dakota, Grand Forks, ND.
Korom, S.F., 1992. Natural denitrification in the saturated zone: A review. Water Resources Research 28, no. 6: 1657-1668.
Manassaram, Deana M., Backer, Lorraine C., and Moll, Deborah M. A Review of Nitrates in Drinking Water: Maternal Exposure and Adverse Reproductive and Developmental Outcomes. Environmental Health Perspectives Volume 114, Number 3, March 2006.
Robertson, W.D., Russell, B.M., and Cherry, J.A. (1996). Attenuation of nitrate in aquitard sediments of southern Ontario. J. Hydrol. 180:267-281.
Schlag, A. J., 1999. In-site measurements of denitrification in the Elk Valley aquifer, M.S. thesis, 104 pp., University of North Dakota, Grand Forks, ND.
Skubinna, A. Paul, 2004. Modeling the hydrogeochemistry of denitrification in the Elk Valley aquifer, M.S. thesis, 141 pp., University of North Dakota, Grand Forks, ND.
Tesfay, T. and Korom, S. F. and. 2006. The relative roles of electron donors in aquifer denitrification reactions: insights from geochemical modeling. 40th annual meeting, North-Central section, the Geological Society of America, University of Akron, Akron, Ohio, April 20-21, 2006.
Trudell, M.R., R.W.Gillham, and J.A. Cherry, 1986. An in-situ study of the occurrence and rate of denitrification in a shallow unconfined sand aquifer. J. Hydrol. 83:251-268.
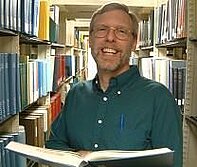
Scott F. Korom
Geology & Geological Engineering
UND